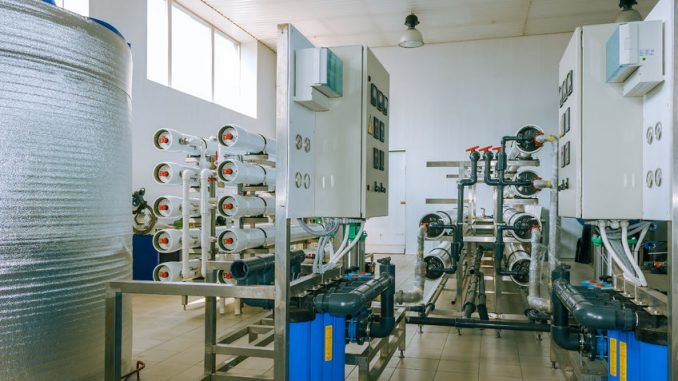
Overview Of Membrane Processes
Membrane processes are commonly used in the food processing industry and there are a vast range of applications in the biological processing industry. Most high-value bioproducts are produced by highly developed fermentation processes coupled to recombinant-DNA technology. The recovery processes are often designed for labile bioproducts which are initially produced in very low concentration levels. The broths are themselves highly complex in their composition so recovery of a specific bioproduct of interest is quite involved. Most membrane processes are classified as non-thermal and offer the opportunity to separate heat-labile materials which would otherwise be damaged by thermal processes.
In downstream processing, a number of steps are required which is characterized as a multi-step operation. Initially solid-liquid separations are involved. These include harvesting and separation of biomass, cell debris removal, collecting precipitates and sterile filtration. The traditional separation techniques for this type of process involve centrifugation and dead-end filtration. These methods are limited unfortunately in their performance because of the nature and behaviour of the biosuspensions being handled. The issues arise from small particle sizes, frequently wide particle size distributions, the high compressability of the solids residues, higher broth viscosities sometimes with non-Newtonian flow behaviour and relatively small density differences (Kroner, 1994).
Commercial Value Of Membrane Processes
In terms of industrial use, it is reasoned that about 20 to 30% of the current 250 million (£) turnover of membranes is used in the manufacturing industry worldwide. The growth in this market is around 7.5% per year. The main applications for membranes are in the dairy industry – about 40% of which over 10% are used in milk protein standardization. Beverages (wine, beer, fruit juices etc.) and egg products (2%) come next. The emerging fields are fruit and vegetable juices and concentrates, recovery from waste streams, co-products as in the recovery and recycling of blood plasma in abattoirs, and then the recovery of technical fluids such as brines and cleaning-in-place solutions.
The global market for membrane technologies for food processing according to bccResearch who are a market research organisation is expected to have increased from nearly $4 billion in 2014 to $4.2 billion in 2015 at a year-on-year growth rate of 6.5%. Going forward, the market is expected to grow at a five-year CAGR (2015 to 2020) of 6.7% to reach nearly $5.8 billion in 2020.
Membrane separation processes can largely be split into three different types. Pressure-driven membrane processes have already been considered but membranes have been used for chromatography and there are electrically enhanced membrane processes. A number of excellent reviews are available which trace the history of application of membrane separation processes (Strathmann, 1981; Zeman and Zydney, 1996).
Types of Formats In Membrane Processes
In membrane separations, the main objective is to optimise for permeate flux versus solute rejection.
Various modules exist such as tubular, hollow fibre, flat sheet and spiral wound formats.
The spiral wound unit in the industrial sense is influenced by a number of factors – operating conditions, the design and arrangement of the spiral wound module in arrays (Schwinge et al., 2004).
It has the advantage compared to other membrane modules of high packing density, low cost per unit area, easy replacement and low energy consumption.
Materials Used For Membranes
Membranes are synthesized from a wide variety of materials. These are either made from organic materials such as polymers or inorganic types like ceramics. Polymeric materials can be hydrophobic, including polytetrafluoroethylene (PTFE), polyvinylidene fluoride (PVDF) and polypropylene (PP), or hydrophilic, including polycarbonate (PC) and modified polyethersulfone (PES) (Mulder, 1996).
The most commonly used membranes for ultrafiltration are cellulose acetate, polyacrylonitrile, polyamide, polysulphone, polyethersulphone and polyvinylidene fluoride.
Polymer membranes are sensitive to both polar and chlorinated solvents, as well as to high oil fractions. This limits their applications. For more diagnostic, analytical and biomedical applications, polyvinylidene difluoride membranes are preferred.
To overcome this, membranes prepared from zirconia show better separation performance. These have higher flux, less fouling and higher oil rejection (Higgins et al., 1994; Yang et al., 1998). Microfiltration membranes are also available in silicon and silicon nitride and various metals and are described as microsieves (van Rijn & Elwenspoek, 1995 van Rijn, 2004). Ceramic membranes have gained wide use especially in the fruit juice processing industry
The surface roughness also influences the separation performance of membranes particularly for ultrafiltration and nanofiltration. For example, membrane fouling is promoted by increasingly rougher surfaces (Evans et al., 2008). The presence of spikes and protuberances on the surface of polyamide membranes for instance may account for fouling started by capture on the membrane surface of matter capture. Membranes based on cellulose acetate for example have smoother surfaces which are less susceptible to fouling.
Housings For Filtration Units
Stainless steel housings are used for cartridge membrane systems. The common materials are AIS 304 and low carbon AIS 316L stainless steels. Best performance is assured with electropolishing. This technology means that surface irregularities are removed. The surface area is reduced though as a result. There is also better resistance to contamination and corrosion.
Calculations For Microfiltration
Flux, more commonly known as permeate flux through a membrane is usually expressed as litres per square metre per hour (in the US, this is gallons per square foot per day for large applications).
Concentration Polarization
Mass transfer across any membrane process depends on a number of factors – porosity, differences in viscosity, changes in the diffusion coefficient and roughness of the membrane wall.
Dimensionless numbers have been applied to define flow through a membrane. Mass transfer equations based on the Schmidt wall number are often employed and have led to what are called Sherwood correlations.
When membranes are used for separations, the molecules being removed for indeed being retained are often more concentrated near the membrane surface than in the main bulk of the retentate stream. The effect reduces the concentration of molecules because flow across the membrane is reduced or there is suppressed passage of compounds across the membrane. This layer occurs even when there is a precipitated cake or gel layer resting on the membrane.
The gel polarization model is based on a simple 1st order differential equation:-
Jv (c-cp) = -D (dc/dx)
where c is the protein concentration within the concentration polarization layer and cp that of the permeate. D is the diffusion coefficient of the protein molecules. Integrating this equation across the concentration polarization layer, the permeate
flux can be given by:
Jv = k ln [(cg-cp)/cf-cp)]
where k is the mass transfer coefficient, k =D/δ, with δ the thickness of the concentration polarization layer. cG is the
protein concentration at the boundary with the gel layer of precipitated solids, and cf the bulk feed concentration.
In the case of high solute rejection, cp→0 and the above equation becomes:-
- Jv = k ln (cg/cf)
or
- Jv = k ln(cg) – k ln (cf)
The rejection of a UF membrane for protein molecules is usually extremely high when a gel layer forms on the membrane surface. The last equation is used for analysing permeate flows through UF membranes in particular.
The effect is most pronounced with laminar flow so to reduce the impact of this phenomenon, turbulent flow is introduced.
The examination of mass transfer and the use of Sherwood correlations was examined a few years ago (Gekas & Hallstrom, 1987).
Dead-End Filtration
Of all the membrane processes, dead-end filtration is probably the most basic of all these processes. The complete feed flow is forced through the membrane and the filtered matter is accumulated on the surface of the membrane. This type of filtration is a batch process. As the accumulated matter grows on the filter, the filtration capacity declines because of clogging and fouling of the membrane. To keep the process running, accumulated material is removed so that the membrane is unclogged. It is a good method for clarification such as cell or large particulates removal. Filter aids are usually needed to provide a porous structure.
Microfiltration
The main purpose of microfiltration (MF) is to clarify liquid streams to remove suspended solids, cells and other hard materials. Separation can best be described as sieving.
One variant on microfiltration, cross-flow filtration (CFF) has been available since the 70s as a viable unit operation in downstream processing. Here, the retentate passes over the membrane where an operating pressure (the transmembrane pressure) causes permeate to cross the membrane barrier.
The technique is especially useful for the recovery of proteins produced by recombinant DNA organisms. No aerosols are produced during the operation and the requirements for safe equipment can be easily fulfilled with considerable savings in costs. The process rivals centrifugation and decanting as the method for recovering cells and particulates.
The general jobs for CFF in downstream processing are bioprocessing:-
- Cell harvesting, cell washing, cell recycling in fermentation
- Cell/cell debris removal and clarification
- Separation and collection of precipitates
- Sterile filtration
The food industry also relies heavily on MF to recover solid materials like yeast or to remove solids from important liquids. It is commonly seen in fruit juice clarification and as an adjunct to centrifugation.
MF exploits symmetric and asymmetric filters all with a pore size that ranges between 0.05 and 10 microns in size.
In some cases, the pore size of the membrane is small enough to prevent fat and high molecular weight (HMW) proteins. The dairy industry regularly uses microfiltration to clarify cheese whey, de-fat and reduce the microbial load of milk (Merin, 1986).
Crossflow filtration is performed in practice in two different ways. There is mechanical agitation which relies on rotating shear filters or by pumping the suspension at high velocity through a filtering channel as in ‘flow-by systems’. The flow-by systems are most commonly applied in bioprocessing and use hollow-fibre, tube or plate and frame modules, similar to that found in ultrafiltration.
Ultrafiltration
Ultrafiltration (UF) is the process of separating extremely small particles and dissolved molecules from fluids. It is often applied to the removal of small impurities as a way of cleaning up product streams before ion-exchange for example.
The principal basis of separation is molecular size although membranes are defined with pore sizes in a range from 0.1 microns to 0.001 microns.
Ultrafiltration can really only separate molecules which differ by at least an order of magnitude in size. Any molecules of similar size are not separated. The molecules range in size from 1K to 1000K molecular weight (MW) depending on the pore size of the membrane employed. This size range covers mainly organic/inorganic polymers, high molecular weight compounds, colloids. In most cases, water and salts including ions (sodium, calcium, sulphates, chlorides) will always pass through these membranes.
In some cases the removal or clearance of small highly charged impurities is poor because of a phenomenon known as electrostatic exclusion. The level of exclusion depends on the respective degrees of charge on both particle and membrane. For example, charged membranes like cellulose and polyethersulphone reject charged particles even when the particles are much smaller than the membrane’s pore size. One thought is to raise the ionic strength of the solution to such a degree that it shields the electrostatic interactions. The phenomenon has been studied by Shao and Zydney (2004).
Another phenomenon which needs examining is the Donnan effect. It is particularly important when concentrating proteins to a high level. Electrostatic interactions between proteins and excipients increases as the protein concentration rises during UF. This is a result of increased protein-charge density and is proportional in practical terms as the protein concentration rises. The interactions create an offset between the diafiltration buffers used in UF and the excipient levels in the final products.
Only low applied pressures are needed to generate high flux rates because the osmotic pressure differential across a UF membrane is extremely small if not negligible. A typical applied pressure might be 50 PSI.
Wide UF membranes, with MWCO ranging between 50 and 100 kDa, can fundamentally be used to recover different types of macromolecules such as suspended solids, carbohydrates, proteins and pectins. UF membranes from 4 to 30 kDa are effective at concentrating high molecular weight components such as tannins, proteins, hydrolysates, and some high molecular weight phenolic
fractions, while tight UF membranes, from 1 to 3 kDa, are highly effective at concentrating low molecular weight compounds such as anthocyanins, low molecular weight PPs, low molecular weight sugars, and peptides); indeed, these membranes are in the molecular limit of the NF process (Galanakis, 2015).
Nanofiltration
Nanofiltration (NF) is a similar method to reverse osmosis and it too is a pressure-driven process which is applied with the separation capabilities of RO and UF membranes. Nanofiltration membranes are relatively loose. It is most often employed to retain proteins and organic materials by removing water and particular types of salt especially monovalent types.
There are times in food processing when a high sodium rejection, typical of RO, is not needed but where other salts such as Mg and Ca (i.e. divalent ions) are to be retained as in dairy whey processing. Like RO, water passes through the membrane leaving higher molecular weight molecules behind. Most nanofiltration membranes are in a spirally wound form.
The separation mechanism involves both steric and electrical phenomena.
The pore-sizes are slightly larger than for an RO membrane but smaller than for a UF type. The pore-sizes for NF are between 100 and 4,000 Daltons. This particular type of membrane system complements ultrafiltration and is used to separate lipids, sugars and large carbohydrates, bacteria, and proteins. The permeate usually contains bivalent salts and undissociated acids.
To understand better and to optimize a process using nanofiltration, the membranes are characterized by using atomic force microscopy (AFM) and scanning electron microscopy (SEM). AFM and SEM analysis allow for visualization of membrane topology such as mean pore distribution, roughness and distribution of separation-layer particles, and morphology (thickness of membrane layers).
The average applied pressure is 1 to 40 bar at a temperature with a maximum of 35ºC.
NF is more economic than evaporation because little heat is involved (Atra et al., 2005).
Typical nanofiltration membranes are composed of polyamide with urea as well as more conventional materials.
Examples of application include the concentration of a lactose syrup (Zhang et al., 2011) but it is most commonly used in the preparation of whey from milk.
Reverse Osmosis (Hyperfiltration)
Reverse osmosis (RO) is a pressure driven process which is designed for the separation of ionic solutes and macromolecules from aqueous streams. The technique is used for dissolved salt and toxic metals removal. Common applications include chloride, fluoride, lead and chromate removal.
RO membranes are characterised by their level of salt rejection. The tightness of a membrane is highest for membranes with a 99.5% salt rejection level. Looser membranes have a 98% and 95% rejection level.
Dialysis
The dialysis process is in contrast to reverse osmosis, a membrane separation technique where no pressure is applied other than that relying on osmotic pressure. The transport of water across a semi-permeable membrane takes place purely on the basis of a colligative property. The movement of water occurs because of a concentration difference between the total number of particles in the retentate versus the dialysate
Electrodialysis
Electrodialysis is a separation process that relies on the movement of ions through ion selective membranes. The movement occurs because of the application of an electromotive force or voltage which is applied across the membrane area. The electrical changes on the ions allow them to be driven through the membranes fabricated from ion exchange polymers. Applying a voltage between two end electrodes generates the potential field required for this.
The technology has been with us for over 60 years on an industrial scale for the production of potable water from brackish water sources. To many it is seen as a relatively novel technology especially in the food industry. It’s now possible to find applications where electrodialysis is combined with bipolar membranes or with ion-exchange resins in a range of processing industries.
The technology rivals many others in separating salts from solution. Rival methods include ion exchange, distillation and reverse osmosis.
A very solid review article covers the benefits and limitations of electrodialysis in a number of applications (Strathmann, 2010). This includes electrodialytic water dissociation or continuous electrodeionization which are two related applications.
Specific applications in the food industry include:-
- Demineralization of whey and milk (Andres et al., 1995)
- De-acidification of fruit juice (Adhikary et al., 1983; 1987; Vera et al., 2003)
- De-ashing of sugar solutions (Boye & Arcand, 2012).
- Recovery of anthocyanins from brining solutions in combination with diafiltration. (Lin et al., 1989)
- One other example is to produce low-salt or even salt-free solutions of soy sauce (Mok et al., 2001), to desalinate brine used in pickling (Pan et al., 1988).
Pervaporation
Pervaporation is a membrane separation process where small volatile molecules are selectively removed from a mixture by diffusion through a semi-permeable polymer sheet. A typical material for this purpose is polydimethylsiloxane. In this method there is a unique difference between the two sides of milieu. One side is liquid -usually an aqueous solution whilst on the other side is a sweep gas or a vacuum. the smaller molecules vaporize and move through the membrane whilst the larger molecules including water are retained. It is the ideal process for removing flavour molecules from a juice for example. It is also a way to recover a concentrated essence by condensing the permeate stream.
Typical examples include alcohol removal from beer and wine, the recovery of aroma from fruit juice, beer and lager, extracts of herbs and flowers etc. It’s also possible to recover aroma compounds from fermentation broths.
Osmotic Evaporation
The technology of osmotic evaporation lends itself very well to membrane separation processes (Alves et al., 2004). The technique is now applied to creating fruit juice concentration because it allows for high levels of concentration of the particulates and large compounds with little mechanical damage and low heat generation.
Applications With Membrane Processes
Membrane processing has found favour in a wide range of industrial categories and none more so than in the food industry. It is extremely popular in the brewing and distilling business. Most firms probably employ some form of membrane system in their processing whether it be for treating raw water to clarification of a liquid to generating an acceptable waste water as effluent.
Milk whey is a valuable byproduct which comes from cheese manufacture. It is a valuable source of proteins for both the food and pharmaceutical industries. In many cases chromatography is the best method to isolate whey proteins on a preparative scale. The early use of membranes is covered by Brans et al., (2004) at the University of Wageningen in the Food & Bioprocess Engineering Group (as then). One of the best reviews covering the recovery of whey proteins which puts membrane processing into context is by Ganju and Gogate (2017).
Very often ultrafiltration is applied for both milk and whey concentration prior to some form of application of chromatography. There are some examples in the literature where whey is concentrated by reverse osmosis or ultrafiltration followed by drying (Jindal & Grandison, 1992) before further processing. Nanofiltration is used for concentrating lactose from a whey.
Acid whey also known as cottage cheese whey, has been partially demineralised and concentrated using nanofiltration (Roman et al., 2009) at a laboratory scale. The membrane system was a flat sheet membrane. The researchers showed a rejection of protein and lactose in the retentate of over 90%.
-Fruit Juice And Sugar Processing
Fruit juice processing is a common application for membrane processing. The methods are often applied to apple juice and orange juice clarification, concentration of vegetable extracts etc. The application is well reviewed (Urosevic et al., 2017). Pomegranate juice processing is one classic example. Probably most fruit juices have been examined especially pulpy types (Cassano et al., 2006; Barreto et al., 2013). Ultrafiltration is used to clarify and concentrate juices and the quality depends on the molecular weight cut-off point of the membrane. The retentate will contain microbes which can pass through an MF membrane as well as proteins, tannins and polysaccharides. The permeate will be a clarified form of the juice devoid of many of its higher molecular weight compounds which actually confer some health benefits.
Pretreatment of the juice is usually needed. One unique example was demonstrated with the clarification of cashew apple juice using enzymes such as tannase and cellulase prior to microfiltration (Matta et al., 2001). The enzymes helped to reduce the impact of fouling materials such as tannins and cellulose. The juice was then microfiltered using a 0.3 micrometre pore size tubular membrane. A certain degree of haze was permitted in the final clarified juice and the flavour and vitamin C content was only marginally reduced by the membrane processing.
Chitosan pretreatment of the juice to bind particulates helps as in the case of the processing with membranes of pequi (de Santana Magalhaes et al., 2018).
A number of studies have combined microfiltration with ultrafiltration as in the clarification of fruit juices such as pineapple (Laorko et al., 2010), kiwifruit (Cassano et al., 2007), pomegranate (Mirsaeedghazi et al., 2010), mosambi juice (Rai et al., 2007).
Sweetener and cane/beet sugar clarification and concentration is a classic process. Sugar solutions always start off brown and are best cleaned using ultrafiltration. One case used ceramic membranes with a 15 kDa cutoff.
- Decolouring of natural sugar solutions especially speciality syrups like maple syrup
- Flavour and fragrance concentration
- Desalting food streams
Molasses is a rough sugar solution which is best clarified using ultrafiltration (Yang et al., 2019).
Beer, wine and cider have are all processed using filtration. Crossflow microfiltration is extensively employed for the recovery of not only brewing yeasts for further process work, but a host of fungi including Aspergillus.
Membranes also make for enzyme reactors (Iorio et al., 1994). Enzymes are globular proteins catalysing a complex myriad range of reactions. Enzymes are effectively contained within living cells which serve as reactors in their own rights. Enzymes are usually obtained by extraction and purification from microbial cells. These whole cells are themselves used as biocatalyst because of their stability however individual enzymes are just as potent.
Nanofiltration is preferable to reverse osmosis because lower pressures can be used but there is a loss of componentry. Some studies have looked at particular fruit juices as model systems. Pomegranate juice is a good example. One particular study looked at a combination of ultrafiltration with nanofiltration where in the latter case, the nominal molecular weight cut-off (MWCO) ranged from 1000 to 4000 Da. As with all membrane systems the quality of the process is judged by high permeate fluxes, a low fouling index and a good separation efficiency of sugars in particular from phenolic compounds (Conidi et al., 2017).
Processing fruit juices also leads onto the recovery of polyphenols.
Water Processing
Whilst purification of water deserves articles in their own right, membrane processes such as RO have been routinely employed for the cleaning up of a variety of water streams. One of the most pertinent is the handling of oil sands process-affect water (OSPW). The return of OSPW back into the environment is of considerable concern because of the presence of persistent organic pollutants which are toxic to aquatic life.
One example includes the use of a Ludzack-Ettinger membrane bioreactor to tackle the OSPW issue (Xue et al., 2016). The choice of a coagulant was important as a way of improving the membrane performance. Napthenic acid appeared the most effective coagulant. This coagulant removed about 25% of the solids content including bacterial cells and the unit was operated for well over a year.
Fouling In Membrane Processes
Membrane fouling in any filtration operation especially cross-flow separation is a key factor which ultimately reduces permeate flux (Wilson, 2018). Fouling is caused by all major classes of biological materials including proteins, carbohydrates and polysaccharides, and lipids. It is most apparent when processing fruit juices and fermentation liquids. Any drop in flux affects both the commercial and economic viability of that operation.
Microfiltration has been studied by Guell & Davis (1996). They looked at the fouling of four different 0.2 micron hydrophilic microfiltration membranes by proteins. These were made of polysulphone, polycarbonate, polyvinylidene fluoride, and cellulose acetate. The proteins were bovine serum albumin (BSA), lysozyme (LY), and ovalbumin (OV). They used electron microscopy coupled to study of loss of flux, total resistance and permeate concentrate over time. Single protein solutions were studied for simplicity and interpretation. With the polysulphone and polycarbonate membranes, so BSA and LY showed just internal membrane fouling over a 3 hour period. Ovalbumin showed that there was an initial phase of internal fouling followed by surface and external membrane fouling.
When it came to binary protein mixtures filtered through polysulphone and polycarbonate membranes, the worst membrane fouling involved those mixtures containing ovalbumin. When it came to a ternary protein mixture, fouling behaviour was different. Fouling there depended on the surface porosities of all the membranes. The lower the surface porosity the more rapid the external membrane fouling.
Fane & Fell (1987) looked at fouling in ultrafiltration modules in great depth. Subsequentially, a number of other articles have been published to try and understand how fouling should be characterised (Cheryan & Alvarez, 1995: Orsello et al., 2006).
Cider and fruit juice are very complex mixtures and contain pectins and protein-polyphenol colloids which foul membranes (Gulec et al., 2017; Zhao et al., 2017; Cai et al., 2018). Wine contains similar types of colloids which present a similar issue (Rayess et al., 2011, 2012).
Membrane fouling which inevitably occurs can be measured using a range techniques – mathematical modelling, scanning electron microscopy (SEM), and atomic force microscopy (AFM) have all been employed. In the processing sugarcane juice, cake formation on the membrane surface appears to be the main phenomenon (Li et al., 2018).
Cleaning Membrane Systems
When a membrane has fouled to such an extent that it is economically unacceptable to keep processing then cleaning is needed. Usually it is the drop in the permeate flux rate which dictates the point when this occurs. A proper cleaning procedure is needed. the frequency and method depends on the feed composition and the process parameters. It is always designed for each system on an individual basis.
A very effective cleaning composition is 1% w/w NaOH and 3000 ppm sodium hypochlorite in solution (Sim et al., 2009). In another involving the processing sugarcane juice with ceramic membranes, tap water, 1.0% NaOH and 0.5% NaClO mixture, and 0.5% HNO3 solution was used (Li et al., 2018). It’s worth noting that even if polyethersulfone (PES) or polysulfone (PSf) membranes are the most chemically stable, chlorine damage occurs (Liu et al., 2000). PVDF membranes are evantually damaged by sodium hydroxide solutions.
Cleaning membranes can be an arduous task. One supplier, PCI Membrane Systems Inc. which is now part of Filtration Group developed a membrane system in the late 90s and 2000 called Reco-CIP so as to treat clean-in-place solutions so cleaning chemicals might be recovered and reused rather than add to the environmental burden. The CIP system was designed to reduce the build up of fats and proteins soils. The membrane tolerates acids and bases used in CIP systems.
A common technique to help remove fouling materials from membranes is to apply ultrasound. The microfiltration of milk is improved by using ultrasound technology which used cavity bubbles to dislodge bound proteins. Other physical methods use pulsed flow, back flushing and in-line turbulence promoters to actively scour the membranes of foulants.
Another approach is to use pulsed flow to improve permeate flux (Jacob & Jaffrin, 2007). In one example, sugar cane juice was clarified using ceramic membranes of 0.05 and 0.10 microns. A number of different strategies were tried but switching the unit off every 5 seconds every 5 minutes appeared to help restore some permeate flux (Sim et al. 2009).
Enzymes are often exploited when protein based gel layers are likely to form. In some cases where protein is a major fouling issue, proteases have been immobilised to the membrane. Papain has been immobilised for example to the active side to minimise gel layer formation during the ultrafiltration of milk (Howell and Velicangil, 1982). They made the claim that there was up to 78% improvement in the cumulative permeate yield when processing either a 0.5% w/w albumin or haemoglobin solution. From their perspective there was a change in the apparent order of the gelation reaction.
Alcalase
It appears that enzyme-cleaned membranes perform better than chemically-cleaned ones when proteins have been filtered. One study on whey protein fractionation showed that enzyme solutions could be reused for consecutive cleaning. There was a 30% loss in activity during each cleaning cycle irrespective of the starting activity of the enzyme solution but at least high cleaning rates were possible (Arguello et al., 2003).
Costing A Membrane Process
When it comes to costs there are a few factors to build in. The most important is the hardware such as the frame and the actual membranes themselves. The type and application very much depends on the type of solution being treated, how much particulate matter it contains, what the specification is for the final product (filtrate) and how the whole process is devised and designed to deal with the changes in flux across the membrane.
Capital costs that are amortized per unit of production decrease with plant capacity due to economies of scale. An industrial average is that a properly maintained membrane system will be of the order of one dollar for every 1000 gallons processed. If the whole facility is badly maintained and not cleaned properly those costs can rise as much as four fold.
Typical costs based on industrial estimates of suppliers would be:
- Filter cartridges: $0.05 to 0.15 / 1000 gallons
- Anti-scaling materials: $ 0.05 to 0.15 / 1000 gallons
- Membrane cleaning with a proper operating system: $0.5 to 1.0 / 1000 gallons for a good operating system
- Membrane cleaning with an improper operating system: as high as $ 4.0 / 1000 gallons
In percentage terms, the capital cost including any automated cleaning facilities is as follows:-
- pumps 30%
- replaceable membrane components 20%
- membrane modules (housings) 10%
- pipework, valves and framework 20%
- control systems 15%
These figures come from Noble and Stern’s book ‘Membrane Separations Technology‘ (1995).
Operating costs vary from application to application and are usually influenced most heavily by capital cost charges. In terms of the operating costs, there are four factors to consider:
- the energy cost of maintaining the system’s hydrostatic pressure and flow rate
- the expected membrane life
- the cleaning regime required
- site-specific factors including labour
The energy consumption for any membrane system is directly related to the flow rate and pressures (including piping and module head-loss) to be maintained which is reflected in pumping fluids around a circuit.
Most microfiltration and ultrafiltration systems operate with low pressures. the energy consumption is associated with maintaining a minimum velocity of around 2 m/s over the membrane surface. Reverse osmosis and nanofiltration operate with higher hydrostatic pressures but lower flow rates.
For a typical membrane system where capital costs are excluded, then operating cost is approximately:
- replaceable membrane components – 35 to 50%
- cleaning – 12 to 35%
- energy – 15 to 20%
- labour – 15 to 18 %
Fouling always leads to added cost and can make these rise by at least 50% if they are allowed to persist.
Few studies have ever examined costings. A UF membrane filtration system for treating drinking water at a pilot scale level demonstrated how an optimised cleaning regime yielded the greatest cost-benefit (Yoo et al., 2018). In this example, permeate flux was the main parameter to optimise and cost savings of just over 25% were obtained using an NaOCl solution. They found an enhanced flux maintenance which improved duration of use of the membrane of 6.3 days compared to 4.3 days if the NaOCl concentration was increased compared to the vendor’s instructions. In their case the total operating cost was $0.1187/m3 of filtrate and the CO2 emission was 112.75 g CO2/m3.
References
Adhikary, S. K., Harkare, W. P., Govindan, K. P., & Nanjundaswamy, A. M. (1983). Deacidification of fruit juices by electrodialysis. Indian Journal of Technology, 21(3), pp. 120-123
Adhikary, S. K., Harkare, W. P., Govindan, K. P., Chikkappaji, K. C., Saroja, S., & Nanjundaswamy, A. M. (1987). DEACIDIFICATION OF FRUIT JUICES BY ELECTRODIALYSIS. 2. Indian Journal of Technology, 25(1), pp. 24-27.
Alves, V. D., Koroknai, B., Bélafi-Bakó, K., & Coelhoso, I. M. (2004). Using membrane contactors for fruit juice concentration. Desalination, 162, pp. 263-270 (Article)
Andres, L.J., Riera, F.A., Alvarez, R. (1995). Skimmed milk demineralization by electrodialysis: conventional versus selective membranes. Journal of Food Engineering, 26, pp. 57–66
Argüello, M. A., Álvarez, S., Riera, F. A., & Álvarez, R. (2003). Enzymatic cleaning of inorganic ultrafiltration membranes used for whey protein fractionation. Journal of Membrane Science, 216(1-2), pp. 121-134.
Atra, R., Vatai, G., Bekassy-Molnar, E., & Balint, A. (2005).
Investigation of ultra- and nanofiltration for utilization of whey protein and lactose. Journal of Food Engineering, 67(3), pp. 325–332. https://doi.org/10.1016/j.jfoodeng.2004.04.035
Barreto, A. G., Cabral, L. M. C., Matta, V. M. D., & Freitas, S. P. (2013). Clarification of camu‐camu pulp by microfiltration. Brazilian Journal of Food Technology, 16(3), pp. 207–215.
Boye, J. I., & Arcand, Y. (2012). Green technologies in food production and processing. Springer Science & Business Media.
Brans, G. B. P. W., Schroën, C. G. P. H., Van der Sman, R. G. M., & Boom, R. M. (2004). Membrane fractionation of milk: state of the art and challenges. Journal of Membrane Science, 243(1-2), pp. 263-272
Cai, M., Lv, Y., Luo, S., Liu, Y., & Sun, P. (2018). Fouling Behavior of Polyphenols during Model Juice Ultrafiltration: Effect of Membrane Properties. Food and Bioprocess Technology, 11(9), pp. 1787-1793 (Article).
Cassano, A., Figoli, A., Tagarelli, A., Sindona, G., & Drioli, E. (2006). Integrated membrane process for the production of highly nutritional kiwifruit juice. Desalination, 189, pp. 21–30 (Article).
Cheryan, M., Alvarez, J.R., (1995). Food and beverage industry applications. In: Noble, R.D., Stern, S.A. (Eds.), Membrane Separations Technology. Principles and Applications. Elsevier Science B.V., Amsterdam.
Conidi, C., Cassano, A., Caiazzo, F., & Drioli, E. (2017). Separation and purification of phenolic compounds from pomegranate juice by ultrafiltration and nanofiltration membranes. Journal of Food Engineering, 195, pp. 1-13 (Article).
de Santana Magalhães, F., Cardoso, VL, & Reis, MHM (2018). Sequential process with bioadsorbents and microfiltration for clarification of pequi (Caryocar brasiliense Camb.) Fruit extract. Food and Bioproducts Processing, 108 pp. 105-116
El Rayess, Y., Albasi, C., Bacchin, P., Taillandier, P., Raynal, J., Mietton-Peuchot, M., & Devatine, A. (2011). Cross-flow microfiltration applied to oenology: A review. Journal of Membrane Science, 382(1-2), pp. 1-19
El Rayess, Y., Albasi, C., Bacchin, P., Taillandier, P., Mietton-Peuchot, M., & Devatine, A. (2012). Analysis of membrane fouling during cross-flow microfiltration of wine. Innovative Food Science & Emerging Technologies, 16, pp. 398-408.
Fane, A.G., Fell, C.J.D., (1987). A review of fouling and fouling control in ultrafiltration. Desalination 62, pp. 117–136.
Ganju, S. & Gogate, P.R. (2017) A review on approaches for effecient recovery of whey proteins from dairy industry effluents. J. Food Engineering 215 December pp. 84-96 (Article)
Galanakis, C.M. (2015) Separation of functional macromolecules and micromolecules: From ultrafiltration to the border to nanofiltration. Trends Food Sci. Technol. 42, pp. 44–63 (Article)
Gekas, V., Hallstrom, B. (1987) Mass transfer in the membrane concentration polarization layer under turbulent cross flow : I. Critical literature review and adaptation of existing sherwood correlations to membrane operations. J. Membrane Sci., 30 (2) pp. 153-170 (Article)
Güell, C., & Davis, R. H. (1996). Membrane fouling during microfiltration of protein mixtures. Journal of Membrane Science, 119(2), pp. 269-284 (Article).
Higgins, R.J., Bishop, B.A., Goldsmith, R.L. (1994) Proceedings of the 3rd International Conference on Inorganic Membranes, Worcester, MA, 10 -14 July, 1994, p. 447
Howell, J. A., & Velicangil, O. (1982). Theoretical considerations of membrane fouling and its treatment with immobilized enzymes for protein ultrafiltration. Journal of Applied Polymer Science, 27(1), pp. 21-32 (Article)
Iorio, G., Calabro, V., Todisco, S. (1994) Enzyme Membrane Reactors. In: Membrane Processes in Separation and Purification. Eds. J.G. Crespo & K.W. Boddeker. Kluwer Academic Publishers. Pp. 149-163
Jindal, A.R. & Grandison, A.S. (1992). Preparation and composition of chhana whey powders using membrane processing techniques. Journal of the Science of Food and Agriculture. 58. Pp. 511-517.
Kroner, K.H. (1994) Cross-Flow Filtration Of Biological Suspensions. In: Membrane Processes in Separation and Purification. Eds. J.G. Crespo & K.W. Boddeker. Kluwer Academic Publishers. Pp. 59-83
Laorko, A., Li, Z., Tongchitpakdee, S., Chantachum, S. & Youravong, W. (2010). Effect of membrane property and operating conditions on phytochemical properties and permeate flux during clarification of pineapple juice. Journal of Food Engineering, 100, pp. 514–521
Li, W., Ling, G., Lei, F., Li, N., Peng, W., Li, K., … & Zhang, Y. (2018). Ceramic membrane fouling and cleaning during ultrafiltration of limed sugarcane juice. Separation and Purification Technology, 190 , pp. 9-24
Lin, S. S., Chiang, B. H., & Hwang, L. S. (1989). Recovery of perilla anthocyanins from spent brine by diafiltration and electrodialysis. Journal of Food Engineering, 9(1), pp. 21-33.
Liu, C., Caothien, S., Hayes, J., Caothuy, T., Otoyo, T., Ogawa, T., (2000) Membrane chemical cleaning: from art to science. In: Proceedings of AWWA Membrane Technology Conference, San Antonio, TX.
Matta, V.M., Campos, D.C.P., Santos, A.S., Cabral, L.M.C., Couri, S. (2001) 88E-19 Clarification of cashew apple juice by microfiltration. 2001 IFT Annual Mtg., June 23-27, New Orleans LA. Abstract
Merin, U. (1986). Bacteriological aspects of microfiltration of cheese whey. Journal of Dairy Science, 69, pp. 326–328
Mirsaeedghazi, H., Emam-Djomeh, Z., Mousavi, S.M., Aroujalian, A. & Navidbakhsh, M. (2010). Clarification of pomegranate juice by microfiltration with PVDF membranes. Desalination, 264, pp. 243–248
Mulder, M. (1996) Basic Principles of Membrane Technology, second edition, Kluwer Academic Publishers, pp. 288–293 (a), pp. 72–77 (b), pp. 421 (c), and pp. 468–469 (d)
Orsello, C.D., Li, W., Ho, C.C., (2006) A three mechanism model to describe fouling of microfiltration membranes. Journal of Membrane Science 280, pp. 856–866
Román, A., Wang, J., Csanádi, J., Hodúr, C., & Vatai, G. (2009). Partial demineralization and concentration of acid whey by nanofiltration combined with diafiltration. Desalination, 241(1-3), pp. 288-295. .
Sablani, S. S., Goosen, M. F. A., Al-Belushi, R., & Wilf, M. (2001). Concentration polarization in ultrafiltration and reverse osmosis: a critical review. Desalination, 141(3), pp. 269-289
Shao, J. & Zydney, A.L. (2004) Retention of small charged impurities during ultrafiltration. Biotechnol. Bioeng. 87(1) https://doi.org/10.1002/bit.20009
Schwinge, J., Neal, P. R., Wiley, D. E., Fletcher, D. F., & Fane, A. G. (2004). Spiral wound modules and spacers: review and analysis. Journal of Membrane Science, 242(1-2), pp. 129-153 https://doi.org/10.1016/j.memsci.2003.09.031
Sim, L., Shu, L., Jegatheesan, V., & Phong, D. D. (2009). Effect of operating parameters and cleaning on the performance of ceramic membranes treating partially clarified sugar cane juice. Separation Science and Technology, 44(15), pp. 3506-3537 (Article)
Strathmann, H. (1981) Membrane separation processes. J. Membrane Sci., 9 (1-2) pp. 121-189 (Article)
_____________(2010) Electrodialysis, a mature technology with a multitude of new applications. Desalination 264 (3) pp. 268-288 (Article)
Tanudjaja, H. J., Anantharaman, A., Ng, A. Q. Q., Ma, Y., Tanis-Kanbur, M. B., Zydney, A. L., & Chew, J. W. (2022). A review of membrane fouling by proteins in ultrafiltration and microfiltration. Journal of Water Process Engineering, 50, 103294.
Urošević, T., Povrenović, D., Vukosavljević, P., Urošević, I., & Stevanović, S. (2017). Recent developments in microfiltration and ultrafiltration of fruit juices. Food and Bioproducts Processing, 106, pp. 147-161.
Vaillant, F., Millan, P., O’Brien, G., Dornier, M., Decloux, M., Reynes, M., (1999). Crossflow microfiltration of passion fruit juice after partial enzymatic liquefaction. Journal of Food Engineering 42 (4), 215–224.
Vaillant, F., Jeanton, E., Dornier, M., O’Brien, G.M., Reynes, M., Decloux, M., (2001a). Concentration of passion fruit juice on an industrial pilot scale using osmotic evaporation. Journal of Food Engineering 47 (3), 195–202.
Vaillant, F., Millan, A., Dornier, M., Decloux, M., Reynes, M., 2001b. Strategy for economical optimisation of the clarification of juicey fruit juices using crossflow microfiltration. Journal of Food Engineering 48 (1), 83–90.
Yu, Z.R., Chiang, B.H., (1986) Passion-fruit juice concentration by ultrafiltration and evaporation. Journal of Food Science 51 (6), pp. 1501–1505
van Rijn, C.J.M. (2004) (Ed.), Nano and Microengineered Membrane Technology, Elsevier Science, Amsterdam, The Netherlands.
van Rijn, C.J.M., Elwenspoek, M.C. (1995) Microfiltration Membrane Sieve with Silicon Micromachining for Industrial and Biomedical Applications, Microelectro Mechanical Systems (MEMS), Amsterdam, The Netherlands, p. 83.
Vera, E., J. Ruales, M. Dornier, J. Sandeaux, F. Persin, G. Pourcelly, F. Vaillant, and M. Reynes. (2003). Comparison of different methods for deacidification of clarified passion fruit juice. Journal of Food Engineering 59 pp. 361–367
Warczok, J., Ferrando, M., Lopez, F., & Güell, C. (2004). Concentration of apple and pear juices by nanofiltration at low pressures. Journal of Food Engineering, 63(1), pp. 63-70.
Wilson, D. I. (2018). Fouling during food processing–progress in tackling this inconvenient truth. Current Opinion in Food Science, 23, pp. 105-112 (Article).
Xue, J., Zhang, Y., Liu, Y., & El-Din, M. G. (2016). Treatment of oil sands process-affected water (OSPW) using a membrane bioreactor with a submerged flat-sheet ceramic microfiltration membrane. Water Research, 88, pp. 1-11.
Yang, G.S. Zhang, N.P. Xu, Shi, J. (1998) J. Membr. Sci. 142 (1998) pp. 235
Yoo, S.S., Chu, K.H., Choi, I-H., mang, J.S., Ko, K.B., (2018) Operating cost reduction of UF membrane filtration process for drinking water treatment attributed to chemical cleaning optimization. J. Environmental Mgt. 206 pp. 1126-1134 (Article)
Zeman, L.J., and Zydney, A.L. (1996) Microfiltration and Ultrafiltration. Principles and Applications, Marcel Dekker, New York.
Zhang, Z., Yang, R., Zhang, S., Zhao, H., Hua, X. (2011). Purification of lactulose syrup by using nanofiltration in a diafiltration mode. Journal of Food Engineering, 105, pp.112–118
Zhao, D., Lau, E., Padilla-Zakour, O. I., & Moraru, C. I. (2017). Role of pectin and haze particles in membrane fouling during cold microfiltration of apple cider. Journal of food Engineering, 200, pp. 47-58.
Read this article and thought what a good piece it was. I believe you need to emphasise the role of membrane distillation much more because this is the technology that is making greater strides in desalination for example than membrane processes like reverse osmosis.